An Atlas of DRAGNs: Glossary
This page attempts to define some of the technical terms used in the Atlas
for the benefit of people who aren't professional astronomers. Unfortunately,
it probably won't make much sense unless you have a scientific or technical
background of some kind. The glossary is subdivided into three sections:
An object so dense that its escape velocity exceeds the speed of light.
According to general relativity,
such an object must collapse to an infinitely dense point, a
singularity. The singularity is surrounded by a surface called
the event horizon, within which objects and information can only
move inwards, quickly reaching the singularity (and being crushed to a point
in the process, of course). Therefore nothing can escape from a black
hole. A technical exception is Hawking radiation, a
quantum mechanical process first described by Steven Hawking, but this
is unimaginably weak for the massive black holes of interest to astronomers.
A physical constant that appears in the theory of general
relativity. It corresponds to a force between particles which
increases with separation, and only has an effect over cosmological
distances, hence the name. Although the constant fits well into the
equations, they are more elegant when the constant is dropped (set to zero).
Einstein originally put the constant in because without it he found that
general relativity predicted an expanding or contracting universe, and
he assumed that the universe must be static. By doing this he missed
the chance to claim the expansion of the universe as a prediction of GR.
To rub salt into this wound, it later turned out that Einstein's model
of a static universe held balanced by the cosmological constant could
never have worked: the balance is precarious and the slightest disturbance
would send the universe into accelerating contraction or expansion.
Not surprisingly, Einstein called the cosmological constant `my greatest
blunder'.
A process hypothesised by Enrico Fermi whereby a small fraction of the
charged particles in space can be boosted up to the high energies of
cosmic rays. The mechanism works on
particles which are already distinctly more energetic than average. Fermi
imagined the high-energy particles scattered from dense clouds in the
interstellar gas, or more correctly from the magnetic field embedded
in the clouds. In these collisions the particle gains energy if the
cloud is moving towards it, and loses if the cloud is moving away, but
Fermi realised that the first case happens slightly more often than the
second, so the particles slowly gain energy.
In a strange coincidence, in 1977 four independent groups of researchers
realised that in shock waves the high-energy particles find that every
scattering is nearly head-on, giving much more efficient acceleration
(called "first-order Fermi acceleration"). According to simplified
calculations, the particles end up with a "power-law" distribution of
energies, very like that of the electrons which actually produce
cosmic synchrotron radiation. It therefore seems
quite likely that the first-order Fermi mechanism is responsible,
although things don't agree so nicely when the calculations
are done more carefully.
Einstein's theory of gravity. Einstein describes a gravitational field
in terms of the "curvature" of space. J. H. Wheeler boiled the theory down
to the slogan
Space tells matter how to move; matter tells space how to curve.
Because of the curvature of space, the various ways we use to define length
end up giving different answers when general relativistic effects are
important, for instance in cosmology, or near
a black hole.
More...
When Einstein proposed the theory in 1916, there was very little evidence
in its favour. The situation is very different today: the predictions of
General Relativity have been tested with high precision in many ways,
in laboratories, in the Solar System, and by observations of distant pulsars.
The results agree very well with General Relativity, showing that it must
be, at the least, very close to the right answer.
For more information, the
Relativity
and FTL Travel FAQ, has quite a lot about GR. A full appreciation of
general relativity involves mastering some difficult mathematics (unlike
special relativity). To be honest, even professional astronomers often
never get around to learning GR properly!
The ratio of the speed of a fluid flow (e.g. a jet) to
the speed of sound, so that supersonic flows have Mach numbers greater than
one. In jets there are two Mach numbers, depending on whether we use
the speed of sound in the outside medium (the external
Mach number) or the speed of sound in the jet fluid (the internal Mach number).
The systematic study of shape or structure. Often used by astronomers
as a pompous synonym for "structure".
Sometimes called the fourth state of matter (after solid, liquid and gas),
a plasma is a gas in which some of the atoms or molecules are ionised,
i.e. their electrons have been stripped off, and are
floating around freely. Strictly speaking, almost all gas in space is
a plasma, although only a tiny fraction of the atoms are ionised when the
temperature is below about 1000 Kelvin. The gas in and
around DRAGNs is much hotter than this, and so is a fully ionised plasma.
The very low densities in space
allow the electrons to travel without much obstruction, so paradoxically
space is an almost perfect electrical conductor. Although charges can
move around freely, averaged over even small volumes (say a million km
across) cosmic plasmas are always neutral (i.e. they contain equal
numbers of positive and negative charges), because the electrical forces which
arise when charges are noticeably separated are enormously strong.
Plasmas in space are permeated by magnetic fields,
A good way to think about cosmic magnetic fields is in terms of field lines.
These behave like rubber bands embedded in the plasma, so that as the plasma
flows the field lines are pulled and stretched along with it. When they
are stretched enough they can pull back on the plasma. Individual electrons
and ions in the plasma feel a magnetic force which makes them travel in
a helical path
around the field lines, so that they can only travel long distances in the
direction along the field. This binds the plasma together so that it
behaves like a continuous medium even when (as in the plasma in DRAGNs)
the individual electrons and ions almost never collide.
Einstein's theory of motion.
The theory is "special" because it describes the special case where gravity
can be neglected. For motions much smaller than the
speed of light, represented by the symbol c, special relativity
gives answers that are almost identical to those provided by "classical
mechanics", i.e. the theory of Newton (which is much simpler to use).
When objects move at speeds near c, classical mechanics gives the
wrong answers and we have to use special relativity.
The most important result is that it takes an
infinite amount of energy to accelerate anything with finite mass to
c, and therefore c acts as a universal speed limit.
When the special features of Einstein's theory come into play, we describe
motion (and the moving objects) as relativistic. For
our purposes, motion faster than about 0.1c counts as mildly
relativistic.
When the motion is highly relativistic, it is not convenient to
talk about speed because everything is moving at almost exactly c.
Instead it is useful to use the Lorentz factor defined
as
.
More...time dilation, beaming, aberration
For more information, see the
Relativity
and FTL Travel FAQ, practically a complete text on Special Relativity.
(Although a real textbook might be easier to use!).
Nature's favorite way of making radio waves, named after the machines
which produce the same type of radiation in laboratories.
Electromagnetic radiation is produced whenever charged particles are
accelerated. The particles which do this best, by far, are electrons
(and their anti-particles, positrons). Different kinds of radiation are
produced by accelerating electrons in different ways; for instance in a
commercial radio transmitter the electrons are accelerated back and
forth in the antenna by a carefully
controlled oscillating voltage, producing radiation at a very precise
frequency.
Synchrotron radiation is produced by all
relativistic electrons in space because they are
accelerated by the cosmic magnetic field.
Although relativistic electrons are very rare, they produce
proportionately much more radiation than normal "slow" electrons, and
so tend to dominate the picture.
The synchrotron radiation produced by a single electron comes out
as a wide range of frequencies peaking at a value set by the electron's
energy and the magnetic field strength. The range of frequencies is spread
out even more because cosmic radio sources contain electrons with a wide
range of energies. The result is a very smooth continuum
spectrum
(see spectral index for more detail).
Another unusual feature of synchrotron radiation is that
it is highly polarized.
The direction of polarization is
perpendicular to the magnetic field in which the electron was moving.
When the field in the
source is uniform, the degree of polarization is around 70%. More often,
we receive radiation from many regions with different magnetic field
directions, and when all these arrive together their polarizations cancel
to some extent: if the field directions were completely random the net
radiation would be unpolarized, but usually there is enough order to give
10 to 30% polarization.
At very high surface brightness, the normal
synchrotron spectrum is modified by the re-absorption
of some of the radiation by the relativistic electrons within the source.
This is called synchrotron
self-absorption. The effect is to cut off the lower frequency
radiation.
A unified scheme is a model which "unifies" apparently different types of
AGN
and/or DRAGN by explaining them as intrinsically similar objects seen at
different angles from their central axis (assumed to be the direction along
which the jets emerge). Occasionally the term is extended to
include models which "unify" different types in more general ways, e.g.
by seeing them as different stages in a life-cycle. In this Atlas we always
mean the more restrictive sense, sometimes called "orientation-unified
schemes".
[Plural: Active Galactic Nuclei, (also AGN)].
To try to summarise what we know about AGN
is to step into a minefield. There is a "standard model"
which everyone agrees is at least partly wrong, but every expert
has his or her own proposal for either fixing it up, or replacing it
with something completely different. To avoid getting too bogged down
in these controversies, the following description is deliberately
vague in many places.
AGN are exclusively found in the centres of large
galaxies; the galaxy
containing an AGN is said to be its host.
The central light-year or so of an AGN contains
an enormous mass, equivalent to at least a million suns, and sometimes
ranging up to a few billion suns. This region also contains something that
shines brightly in the part of the electromagnetic spectrum
from the ultraviolet through to X-rays.
There is pretty good (but disputed) evidence that all this is actually
concentrated on a much smaller scale, sometimes less than a few light-days
across. Certainly the X-rays originate in an astronomically tiny
volume, smaller than our own solar system.
To put this into perspective, within a light-year of us there is just
one sun (ours), and the host galaxies
are typically 100 thousand light-years across or more.
The thing (if it is one thing) at the heart of an AGN is often called
the "monster". If general relativity is correct
it seems almost inevitable that nearly all the mass of the monster
is contained in a spinning black hole.
Black holes don't radiate, by definition,
so the radiation from an AGN is believed to come
from gas clouds falling into the hole. In a way, the monster works as a sort
of engine, fuelled by matter falling in (accreting, in the
jargon). To be more precise, the gravitational potential energy of the
accreted stuff is ultimately converted to radiation, and to kinetic
energy in the form of jets. We don't know just
how this happens, although there are dozens of competing theories.
At larger distances from the centre swirl clouds of gas and dust which
are lit up and heated by the central heart, producing the characteristic
emission lines and infra-red radiation. The outermost regions can be
imaged from Earth in some of the nearest AGN, but mostly AGN appear just
as points of light.
Perhaps the weirdest feature of AGN is that they can produce narrow
jets of material streaming outwards from the centre.
The jets are usually
produced in pairs, pointing in opposite directions; occasionally there
only seems to be one jet. These jets can sometimes be "seen" directly by
radio telescopes, but more often we
deduce their existence because far outside the galactic nucleus they
produce a DRAGN. In one sense, we should not be too surprised to
find jets, since in every other case where accretion is
thought to occur in astronomy, jets are also common. These include
young stars forming out of gas clouds, and binary stars where matter
is falling from one of the pair onto the other. Unfortunately, we don't
know how jets are produced in any of these circumstances!
Astronomers have defined a dozen or so major types of AGN, each with a
different overall pattern of emission. Some of these "types"
are only apparently different; for instance,
if a thick dust cloud lies between us and the
centre, it can block out the radiation at frequencies between
visible light and X-rays,
so that we only see the radio, infra-red, and high-energy radiation,
plus the emission lines from outlying clouds. ---Picture here---
In at least some cases, the dust in the nucleus forms a thick ring around
the centre, like an american doughnut (the technical term is torus).
Then the appearance of the AGN depends radically on whether we view it
side-on, so the centre is hidden, or face-on, so the centre is visible.
Based on rather circumstantial evidence, jets
are thought to emerge from the AGN at right angles to the dust torus.
The various types of AGN can roughly be categorised into two major
divisions: radio-loud and radio-quiet. In each division there is a huge
range of luminosity, from AGN that are so weak they are barely detectable
against the light from central stars of their host galaxy, to quasars which
are a hundred or more times brighter than all the stars in their hosts
put together.
Radio-loud AGN
These always produce jets, which are very fast, at least at first.
In many, perhaps all, cases, the flow speed is
close to the speed of light, and we can see many effects predicted by
the theory of special relativity.
The power of the jets (i.e. the kinetic energy flowing down them per second)
roughly matches the luminosity (i.e. the energy in electromagnetic radiation
flowing out from the AGN per second). The best estimate (quite uncertain)
is that the jet power is rather larger. Radio-loud AGN are generally
are found in elliptical galaxies, almost never in
spirals.
Radio-quiet AGN
Many of these also have detectable jets,
but they are thousands of times weaker relative to the electromagnetic
luminosity. Because the jets are so weak, we know little about them.
Radio-quiet AGN are generally found in spiral galaxies,
although some of the most luminous ones have elliptical
hosts.
The obvious differences between spirals and ellipticals occur on scales
many times larger than that of the AGN, and we have no real idea how AGN
"know" which type of galaxy they are in.
Distances to galaxies are found by a long chain of arguments called the
cosmological distance ladder.
- A scale model of the solar system can be constructed from observations
of the motions of the planets in the sky. All distances are known in
terms of the radius of the Earth's orbit, the
Astronomical Unit, (AU). Copernicus made the first
roughly accurate solar system model, using data taken in ancient times,
in his famous De Revolutionibus (1543). Modern models are
exquisitely accurate.
- The actual distances to most of the planets can be measured by radar,
and since we also know the distance to them in AU, the length of the AU can
be found (to nine significant figures!).
- Distances to nearby stars can be found by various geometrical methods.
The simples is via
their annual parallax, i.e. their apparent change of position in the sky
caused by the motion of the observer on Earth around the sun. The best
precision is now about three significant figures in a handful of cases.
More usefully, the final results of ESA's
Hipparcos
satellite (released in April 1997) give the parallaxes of around 10,000
stars to within few percent. Before Hipparcos, annual parallaxes were not
as important as some more subtle geometric methods, but the new data will
change the situation completely.
- Stars of similar type have similar luminosities. Thus if we know a
star's type (from its colour and/or spectrum) we can find its distance
by comparing its apparent with its absolute magnitude;
the latter derived from geometric parallaxes to nearby stars. Unfortunately
nearby stars are not very bright in absolute terms, so we cannot see
distant versions very far away (certainly not in other galaxies).
- Distances to the super-bright stars that can be seen in
other galaxies (especially Cepheid variables and RR Lyrae stars) are found
by searching for distant star clusters in our Galaxy that contain both
a Cepheid (say) and some fainter stars whose absolute magnitudes are
known directly.
- Distances to the nearest galaxies are found using Cepheids, RR Lyraes,
etc. The Hubble Space Telescope is now finding Cepheids in galaxies
about ten times more distant than was possible from the ground.
- For more distant galaxies, we need objects even brighter than Cepheids.
Examples are supernova explosions, planetary nebulae, and globular star
clusters. The absolute magnitudes for such things can't be easily found
in our own Galaxy, so they are measured in nearby galaxies (or clusters
of galaxies) with Cepheid or similar distances.
- At the furthest limits, only whole galaxies are detectable.
Galaxies come in a very wide range of luminosities, so we need a way
to find their luminosity before we can get their distance. Various
methods exist. For instance galaxy luminosity is related to the
speed of internal motions; most radio galaxies seem to have similar
luminosities; the range of brightnesses in
clusters of galaxies do not vary much from one
cluster to another.
At every step of the distance ladder, errors and uncertainties creep in.
Each step inherits all the problems of the ones below, and also
the errors intrinsic to each step tend to get larger for the more distant
objects; thus the spectacular precision at the base of the ladder degenerates
into an uncertainty of a factor of several at the very top.
To find Hubble's Constant, the ratio of the
cosmological recession speed
to the distance, we need to go up to Step 7
of the ladder. This is because we can only measure the sum of the
recession speed and the random motion of a galaxy, and so we need to
go far enough away that the the random motions are small compared to the
recession speed.
More details of individual methods are given in Ned Wright's
The ABC's of Distances.
A good book describing the Distance Ladder in detail is
Rowan-Robinson (1985)
(although a lot has happened since it was written).
Far from being distributed at random through space,
galaxies and dark matter
seem to form in a network of sheets and
filaments (the
Las Campanas
Redshift Survey shows this in spectacular detail). Where sheets
intersect, there can be enough galaxies in a small region for gravity to
overcome the expansion of the universe,
and the galaxies form a cluster, each orbiting in the combined
gravitational fields of the others (and the inevitable dark matter).
As well as galaxies and dark matter, clusters contain hot diffuse
gas, the intra-cluster medium. Because it is so hot,
the ICM emits X-rays, and so can be mapped by X-ray telescopes. In large
clusters, the ICM contains as much or more mass as the stars in the
galaxies (but both are out-weighed 10:1 by the dark matter).
Elliptical galaxies are unusually common in clusters,
perhaps because ellipticals are produced by galaxy mergers, which are almost
inevitable when so many galaxies are so close together.
As DRAGNs are usually hosted by ellipticals, they
too are often found in clusters.
The nearby rich clusters were systematically cataloged by George O. Abell,
and are now known by their Abell catalog numbers. Ray White
maintains a gallery of
Galaxy Cluster
Mug Shots featuring optical and X-ray images of some of the most
interesting examples.
Any type of electromagnetic emission which produces radiation over a
relatively wide range of frequencies. c.f. line emission.
The central region of the intra-cluster medium in
clusters of galaxies can sometimes lose
so much energy via X-ray radiation that it cools noticably over the lifetime
of the universe. Like any gas that cools, the pressure goes
down. Now, the ICM is prevented from collapsing under gravity by its own
internal pressure, so the slow loss of pressure from the centre causes the
ICM to gradually contract; this phenomenon is called a cooling flow.
Actually the "flow" is far too slow to measure; it has to be inferred
indirectly from theory.
Cosmic rays are really charged particles such as protons, alpha-particles
(i.e. helium nuclei) and electrons, travelling at almost the speed of
light, c. From the theory of special relativity,
cosmic rays carry a very high energy (tending to infinity as the speed tends
towards c). This energy is much larger than their
rest-mass energy (mc ²), and so they are also known as
high-energy or relativistic particles.
Galaxies are often described as huge collections of stars. This is about
as accurate as describing a city as a huge collection of street-lights:
stars are certainly the most obvious feature of galaxies when see far away
in the dark, but they represent a fairly small fraction of the total mass,
90% or so of which consists of the famous dark matter, whose nature
is one of astronomy's biggest mysteries. As well as stars and dark matter,
galaxies contain gas and dust in the
inter-stellar medium between the stars. The may
also contain an active galactic nucleus at their centre.
Galaxies come in a wide range of sizes (meaning diameter,
brightness,
and mass, all of which roughly go together), ranging from
tiny dwarfs with only a few million stars,
through normal galaxies
like our own Milky Way, with a hundred billion stars, to the
trillion-star cD galaxies
that sit at the centres of the great clusters of
galaxies. As is often
the case in nature, the smallest are the most common; on the
other hand the size of the larger galaxies more than makes up for their
rarity, so that a typical star is likely to be in a galaxy with a size
approaching that of the Milky Way or larger, and large galaxies are also
the easiest to find in the sky, due to Malmquist
bias.
Normal galaxies come in two basic types: spiral
and elliptical.
NGC 2997 © Anglo-Australian Observatory.
Photo by David Malin
|
The most common type of normal galaxy. They are
distinguished not so much by spiral structure as by a disk of stars and gas
all orbiting the galaxy on nearly parallel tracks. Spiral patterns are
"ripples" in the disk.
Much of the gas in the disk is in the form of
cool dense clouds, easily visible in edge-on galaxies because dust in the
clouds obscures the starlight behind, causing a dark "dust lane" to cross
the galaxy.
NGC 891 © IAC/RGO/D. Malin
|
Where conditions are right, the gas clouds can contract under
the force of their own gravity to form clusters of new stars. Therefore
the disks of spiral galaxies are home to many newly-formed stars, the
most massive of which give the galaxies a characteristically blue tinge.
As well as the disk, spirals contain a "bulge" and "halo" of stars moving
on more random orbits.
M 87 © Anglo-Australian Observatory.
Photo by David Malin
|
Normal ellipticals are quite rare through most
of space, but form the majority in big
clusters of galaxies, and the
most massive galaxies are ellipticals (or the similar cD's)
in cluster centres.
Elliptical galaxies are rather featureless, and difficult to show in
pictures because the brightness
falls off so quickly with distance from
the centre that you usually end up with the middle over-exposed and the
outer parts too faint to see. However you work the exposures,
the shape is always elliptical, hence the name.
The orbits of stars in ellipticals are in random directions, and often
very elongated, taking them close in to the centre and then far into
the outskirts.
It was once thought that ellipticals were oblate spheroids, i.e.
shaped like a hamburger bun, and that they kept this shape because they
were slowly rotating. We now know that they are often prolate,
like an american football, or even triaxial, meaning they have
different "diameters" in all three directions. They hardly rotate at
all; instead the shape is maintained because the stars move faster in
the direction of the long axis, and so can get further from the centre
before gravity turns them back. Another myth about ellipticals is that
they contain no gas. In fact they only lack the cold gas found in
spirals; their hot inter-stellar medium can contain
quite a large mass (though usually rather less than spirals). The lack
of cold gas has one crucial effect: stars cannot form any longer
in elliptical galaxies; instead all the stars were formed in one go
at the same time as the galaxy itself, which probably happened a billion
years or so after the big bang. Because bright blue stars have short
lives, there are none of these left in ellipticals, which have the
reddish colours of long-lived stars. An important exception to these
comments is that many ellipticals, especially those containing AGN, have
a "mini-disk" embedded in their centres, which can contain cold gas
and young stars.
These term comes from a classification scheme for galaxies that never really
caught on, except for these two (especially cD).
They are very similar to ordinary ellipticals,
but in the brightness declines rather
more slowly with distance from the centre, making it look as if they
have a diffuse halo ("D" stands for "diffuse"). For arcane reasons
the "c" means the galaxy is extremely luminous.
cD's are supergiant galaxies which sit at the centres of rich clusters of
galaxies; they are usually several times bigger and brighter than any other
galaxy in the cluster. D galaxies are somewhat smaller versions of cDs.
In DRAGNs with one-sided jets,
or twin jets in which one is distinctly brighter than the other,
the lobe containing the (brighter) jet
is almost always
less depolarized than the lobe on the other
("counter-jet") side.
Unified schemes explain the effect neatly, as
follows. The asymmetry between the two jets is caused by
relativistic beaming, so the brighter jet is coming
towards us and hence on the nearer side. The two lobes are embedded in
the interstellar medium or halo of the host galaxy,
and there is more of this stuff in front of the further lobe than the
nearer. As the radio waves from the lobes travel through this material
they are affected by Faraday rotation which causes
the depolarization. Hence the further lobe, on the counter-jet side,
is more depolarized.
The effect is named after its discoverers,
Robert A. Laing and Simon T. Garrington.
An electromagnetic emission process which produces radiation at a number
of specific frequencies, c.f. continuum emission.
It is so-called because when the light is analysed with a spectrograph,
line emission shows up as bright lines crossing the
spectrum.
--picture?--
Line emission is generally produced by fluorescence, in which
atoms or molecules are "excited" to a high energy state by absorbing
ultraviolet radiation, and then "decay" to their "rest state" via a
series of quantum jumps. In each quantum jump, a photon is emitted with
a specific frequency which is determined by the quantum properties
of the atom. The frequency of the photon when received on Earth
is affected by the Doppler effect because
the emitting atom is moving relative to us. Of course we actually
receive the accumulated radiation from large numbers of atoms moving
with different speeds, so emission lines have a finite width, which
are usually specified in terms of the equivalent range of speeds.
--More: permitted & forbidden lines, notation--
[Plural: media.]
Generic name for the stuff that fills space, a very dilute gas composed
mainly of hydrogen and helium.
When the temperature exceeds a few thousand Kelvin, the gas is ionised
and is therefore technically a plasma.
Important examples of cosmic media are:
- Inter-stellar medium (ISM)
- The medium between the stars within a galaxy.
In spirals the ISM is a complex soufflé of
hot (million Kelvin) and cool (3 to 100 Kelvin), gas, where the cooler
regions have higher density so that the pressure is roughly constant.
In ellipticals the ISM consists almost entirely of
"hot" gas (roughly ten million Kelvin) which extends seamlessly into
a gaseous halo surrounding the galaxy.
- Intra-cluster medium (ICM)
- The hot gas (several tens of millions of Kelvin) filling groups and
clusters
of galaxies. In big clusters, the ICM can contain more material than all
the galaxies put together.
- Inter-galactic medium (IGM).
- The gas filling the regions between clusters of galaxies. We don't
know whether there really is a proper IGM, or whether the gas just gets
thinner and thinner as you go away from one cluster, until you reach
the far outskirts of the next.
The common name for our own Galaxy (which is just greek for Milky Way!).
Astronomers usually just call it the Galaxy, with a capital G to distinguish
it from other galaxies in the universe. The Milky
Way is a large galaxy of spiral type, and our sun is in the disk, roughly
25,000 light-years (8 kpc) from the centre. The disk is visible
as the faint band of milky light that circles the night sky, which is
made up of myriads of distant stars.
A starburst galaxy is a galaxy that is undergoing a
sudden burst of star formation.
This has a big effect on its luminosity because the most
massive and luminous stars are very short-lived and so the number of them
in a galaxy depends on the rate at which stars are being formed, rather than
the total mass of the galaxy. The luminosities at radio and far infra-red
wavelengths are particularly boosted in starbursts, and as a result
starburst galaxies are the second-brightest type of extragalactic radio
source, after DRAGNs.
Technically, a starburst galaxy is defined as one which is making stars
so fast that it would convert all its gas into stars in a much shorter time
than the age of the universe; so that we really are looking at a
"burst" which must have started in the recent past. On the other hand,
the lower rates of star formation which go on continuously in
spiral galaxies produce radio emission in exactly the
same way as in starburst galaxies. For this reason
Starburst emission has come to mean all radio emission
produced as a by-product of star formation, whether in genuine starbursts
or not.
More details about starburst galaxies are provided by the
Jodrell Starburst research
group.
The technique of combining the signals from a collection of individual
antennas or telescopes to provide an image with a
resolution
equivalent to a single telescope with a size roughly equal to the maximum
distance between the individual antennas. This may be quite large,
e.g. 217 km for MERLIN, and up
to the size of the Earth for VLBI.
The technique is briefly explained in the
MERLIN User's Guide.
The business of observational astronomy boils down to measuring the
brightness of celestial objects. Unfortunately, the English word
"brightness" covers three quite different concepts, each of which
covers several subtle variations.
Luminosity
When we describe Eta Carina as the brightest star in the Galaxy, we
are talking about luminosity. This is a measure of how bright things
are intrinsically.
It can be measured in Watts, or Watts per Hertz
to describe the luminosity at a specific frequency. For historical reasons
in optical astronomy absolute magnitude is used, and radio
astronomers quote the power, which is the luminosity
per steradian (multiply by 4 pi to get back to luminosity).
Flux Density
When we describe Sirius as the brightest star in the sky, we are talking
about flux density. This is a measure of how bright things seem to us
from our platform on Earth. It depends both on the luminosity and the
distance, since distant objects appear fainter.
Radio astronomers measure this in units
of Jansky, optical astronomers use
apparent magnitude.
Intensity (or Surface Brightness)
This is what we mean when we say that the centre of a galaxy is brighter
than the outer regions; an image is essentially a map of intensity.
It measures the flux density we receive, not from the object as a whole,
but from each unit area of the sky (technically, solid angle).
The flux density of an object is thus the product of its intensity
times its solid angle.
As radio waves travel through the inter-stellar medium,
their plane of polarization slowly rotates, with the
amount of rotation increasing with the square of the wavelength.
This phenomenon is known
as Faraday rotation, since Michael Faraday discovered the analogous effect
in light passing through certain materials. The rate of rotation
depends on the density of electrons and the strength and direction
of the magnetic field
through which the radio waves travel, and so Faraday rotation can be used to
investigate these quantities. The total amount of Faraday rotation
affecting the radio waves from a source is called the Rotation Measure
(RM).
Unit of flux density, named after Karl Jansky, who
first discovered extra-terrestrial radio waves (from the Milky Way) at
Bell Telephone Laboratories in Holmdel, New Jersey, in 1932.
1 Jy = 10-26 Watt Hertz-1metre-2.
In the second century B.C., the greek astronomer Hipparchus made a catalogue
of stars, and divided them into five "magnitudes", with stars of the
first magnitude the brightest, and the fifth magnitude the weakest. In
the 19th century it became possible to measure accurately the relative
brightness of stars. Tragically, rather than starting anew with a
sensible system, astronomers followed a proposal by Pogson which re-defined
magnitudes in such a way that most of the traditional magnitudes of stars
stayed roughly the same. The awful formula that resulted has become one
of the initiation rituals of modern astronomy, and is therefore not
described here. Suffice it to say that we are stuck with a logarithmic
scale on which the numbers get smaller as stars get brighter, so that
the brightest stars even have negative magnitudes. These are actually
measures of flux density, known as
apparent magnitude in this context. The equivalent
measure of luminosity, absolute magnitude, is the apparent
magnitude an object would have if seen from a distance of
10 parsecs.
A measure of the amount of obscuration between an emitting region and the
observer. The optical depth is usually represented by the greek letter
(tau), and is defined as:
where
is the observed intensity and
was the intensity at the emitting region. This definition
also makes optical depth proportional to the amount of obscuring stuff
along the line of sight.
The optical depth actually depends on the frequency of radiation,
with different parts of the electromagnetic spectrum being affected by
different kinds of "stuff".
For instance, blue light is strongly affected by interstellar dust, so
dust clouds have high blue-light optical depth; on the other hand radio
waves are unaffected by dust so the radio optical depth of dust clouds
is zero.
Electromagnetic waves consist of vibrating electric and magnetic fields,
with the direction of vibration perpendicular to the direction of motion
of the wave. If the direction of vibration remains steady with time, the
wave is said to be 100% linearly polarized in that direction. If the
direction of vibration rotates at the same frequency as the wave, the
wave is said to be 100% circularly polarized. Most naturally occurring
electromagnetic waves have a direction of vibration that jiggles around
at random: these are said to be unpolarized.
Intermediate states, where there is some jiggling around
an average direction, are said to be partially polarized; the amount
of order is specified by the degree of polarization which ranges
from 0 to 100%.
Synchrotron radiation is unusual as a natural source
of highly-polarized radiation.
Polarization is affected by Faraday rotation. In an
extended source such as a DRAGN, the polarization from different regions
may be rotated by different amounts, which can depolarize
the source. The rotation, and hence depolarization, is negligible at short
wavelengths, and becomes progressively more important at longer wavelengths.
The direction of an "arrow" on the sky, for instance the long axis of
a DRAGN, is given by its Position Angle (PA), which is
zero if the direction is due north and increases as the arrow rotates
to the east, i.e. to the left, if north is at the top of the
image, since you are looking up.
All images are blurred to some extent; otherwise they would contain
infinitely fine detail and hence an infinite amount of information.
The amount of blurring is technically called the resolution of
the image, with high resolution meaning little blurring. This can
be quantified by defining the Point spread function, which
is the pattern produced by the spread-out light from a single point.
For historical
reasons, radio astronomers call the point spread function the
beam.
The beam on the images in this atlas is a simple
pattern technically called a "Gaussian"; its "size" is characterised by
the full-width at half maximum (FWHM).
The maximum resolution available from a telescope is set by the
phenomenon of diffraction, and gives a FWHM approximately equal to
the ratio of wavelength to the diameter of the telescope. Since radio
waves have very long wavelengths (e.g. 21 cm for most of the images in
the Atlas), they have to be very large to achieve high resolution. This
is usually achieved by using the technique of Aperture
Synthesis.
Gaussian Beams
| Linear LUT | Logarithmic LUT. |
Intensity profile. |
At typical scale in the Atlas |
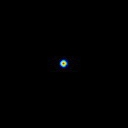 |
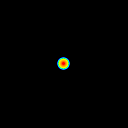 |
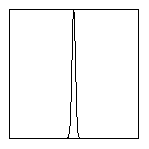 |
magnified × 10 |
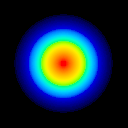 |
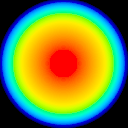 |
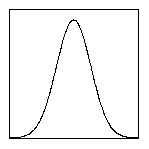 |
The flux density
of synchrotron radio sources, including DRAGNs,
declines with frequency
roughly as a power law, so the spectra appear as straight lines on a log-log
plot of flux density vs. frequency. The spectral index is the (negative)
of the slope on such a plot, i.e. it is defined by
,
where
is the flux density at frequency
, and α is the spectral index.
Insert radio spectrum here!
When the spectral index is zero, the flux density is independent of
frequency, and the spectrum
is said to be flat.
The spectrum of an object is a graph of its brightness
at each wavelength of light, versus wavelength. Sometimes, instead of
wavelength, frequency or photon energy is used; all three graphs are
equivalent. Here 'light' is used as a shorthand for electromagnetic
radiation of all kinds, from radio through to gamma rays.
You can think of an astronomical image as a map of the radiation received
from some small region of the sky. The coordinates of the map therefore
correspond to angles on the celestial sphere. This means that the
directly-measurable "sizes" and "areas" of astronomical objects are actually
angles and solid angles; to convert to real lengths we have to find the
distance, which is usually not known very accurately. Astronomers still
use the units of angles invented by the ancient Babylonians, in which
the 360 degrees of the circle are each divided into 60 minutes of arc,
or arcmin,
and each minute of arc is divided into 60 seconds of arc,
or arcsec.
Since the Babylonians stopped here, fractions of an
arcsec are quoted using decimal notation, and even S.I. prefixes, hence
milliarcsec, microarcsec. Radians
rarely makes an appearance in astronomy. However, solid angles are often
quoted in steradians; square arcsec is a popular alternative.
If this seems needlessly complicated, check out the way
coordinates on the sky are specified.
Since metres are such a pathetically small unit of length compared to
the distances between stars and galaxies, astronomers have invented
other units of length:
- Light-year
- 9.46×1015 metres.
The distance light travels in a year.
- Parsec (pc)
- The preferred unit of astronomers, apparently adopted in the late 19th
century because light-years were becoming too widely used by the general
public! Roughly the distance to the nearest star.
1 pc = 3.26 light years = 3.086×1016 metres.
- kiloparsec (kpc)
- A thousand parsecs. A useful unit when describing galaxies, which can be
anything from a few kpc to 100 kpc in size.
- Megaparsec (Mpc)
- A million parsecs. Typically used to give distances to galaxies.
A visibility is the technical term for the datum produced by a radio
interferometer or aperture synthesis array. It
is a measure of one Fourier component of the region of sky being observed.
Very Long Baseline Interferometry: a form of Aperture
Synthesis in which the individual telescopes are not directly connected
together, but instead record their data on magnetic tape. At a later date,
the tapes are shipped to a central "correlator" where they can be
played back together and the signals combined. The
advantage of this system is that the telescopes in the array can be
arbitrarily far apart, (often on different continents) and so
the technique provides the highest resolution
images in astronomy, with beamwidths in the range 0.1 to 10
milliarcsec (depending on the radio frequency).
Last modified: 1997 March 15
J. P. Leahy
jpl@jb.man.ac.uk